|
|
|
 |
From
Embryo to Ethics |
 |
|
|
|
 |
 |
 |
 |
 |
 |
 |
 |
 |
When
the neural tube closes early in the development of the
embryo, some of the cells on its dorsal side separate
themselves from this tube to form the neural
crest. These cells then migrate throughout the embryo.
Those in the rostral portion of the crest form the cranial
nerve ganglia, the parasympathetic ganglia, the Schwann
cells, etc. The cells in the caudal portion give rise to
the dorsal root ganglia, sympathetic ganglia, intestinal
ganglia, etc.
Though the migration route of these cells is determined by
their position along the neural crest, the specific final position
of the neurons is not determined at the start of migration
but is instead strongly influenced by the environment encountered
in the course of it.
Experiments with neural crest cells isolated and grown in
vitro have also shown that the choice of the neurotransmitter that
a neuron will synthesize is not completely preprogrammed either.
On the contrary, the environment in which the neuron develops
will affect the expression of its capabilities
for synthesizing neurotransmitters. Thus, some extrinsic
chemical factors are needed to activate or deactivate the genes
that control certain neurotransmitters. |
Researchers have developed
special strains of mice with certain mutations that help
provide a better understanding of neuron migration. One of
the best known examples of these strains is the weaver mouse, which
has a tentative, trembling posture. In this mutant mouse,
the granular cells of the cerebellum die before they can
migrate in the inner granular layer and form their parallel
fibres. Contrary to what was initially believed, this mutation
does not seem to affect the radial glia that guide the migration
of these granular cells. Instead, it seems to affect a component
of a potassium channel in the granular cells themselves.
The result is catastrophic for all of the circuits of the
cerebellum and leads to the motor problems observed in this
mutant mouse.
|
The time at which a
neuron is generated helps to determine its final position
in the brain and therefore influences all of its future connections.
The first neurons generated within a given proliferative
unit are located in the deepest layers of the cortex. Because
these deeper layers will thus already be occupied, the neurons
generated later will migrate farther, to constitute layers
closer and closer to the surface of the cortex. It therefore
follows that neurons that occupy the same layer are approximately
the same age. |
The first neurons form
at the end of the 4th week of gestation. Starting on the
33rd day, differentiated development of the spinal cord and
the brain can be observed. Between the 2nd and the 5th month,
the formation of neurons reaches its peak; it is completed
a few months after the baby is born.
The rudimentary structures of the cortex begin
to appear after 6 weeks. Around the 10th week, the neurons
begin to form
connections. This is the start of the communication
network that will enable the individual to generate appropriate
behaviours. |
|
|
HOW STEM CELLS FORM
NEURONS |
|
Starting with its very first mitosis, the zygote begins
a long process of cell
differentiation. As it proceeds through the various phases
of its development, the potentialities of its various cells gradually
become more limited.
The totipotent
zygote, which is capable of producing the entire organism,
will first divide into pluripotent cells that do not have this
ability but can nevertheless produce all the tissues of the
organism. Next will come multipotent cells, which can produce
various cells within a particular tissue. And last will come
specialized cells.
Since all of the cells in a person’s body contain the
same genetic inheritance from that person’s parents,
the factors that determine the location, morphology, and function
of a future neuron are necessarily linked not only to the presence
of specific genes but also to their expression and, ultimately,
to their products: special proteins called transcription factors (follow
Advanced Tool Module link to the left).
In the layers of the telencephalic
vesicles that will form the cortex, a veritable cellular
choreography takes place during the proliferation phase that
produces the neurons and glial
cells.
|
|
The cell
proliferation phase begins when a cell in the ventricular
zone of the neural tube sends an extension through its marginal
zone to its outer surface, at the pia mater. The nucleus of the
cell itself then migrates into the marginal zone along this extension
while replicating its DNA. Next the nucleus, which now contains
two copies of its genetic material, heads back into the ventricular
zone. The cell then retracts its extension and divides in two.
The fate of the resulting two daughter cells depends on many factors.
The first of these is the orientation of the plane of cleavage
during cell division. If the cleavage takes place in the vertical
plane, the two daughter cells will remain in the ventricular zone
and divide again. But if the cleavage takes place in the horizontal
plane, then the daughter cell that is farther from the ventricular
zone will no longer divide and will begin migrating to its ultimate
location. The other daughter cell will remain in the ventricular
zone and continue dividing.
Hence, during the early stages of development, vertical cleavage
predominates, to increase the population of neuronal precursors.
Later, the pattern reverses, and horizontal cleavage becomes the
rule. In this latter case, the uneven distribution of some transcription
factors in the parent cell contributes to this differentiation
(see the explanation below the following diagram, and follow the
link below for a discussion of more recent results that call this
hypothesis into question).

|
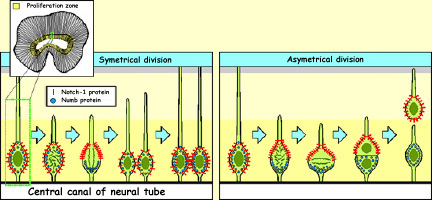
If certain transcription factors
are not uniformly distributed in the cell before it divides,
then when division takes place, the cleavage plane may be such
that one of the daughter cells receives all of a given transcription
factor while the other receives none. This difference will
affect their futures.
One such case involves two kinds
of proteins, Notch1 and Numb, which migrate to different
poles of the neurons in the ventricular zone. When these
neurons divide vertically, both proteins are distributed
symmetrically between the two daughter cells. But if the
neurons divide horizontally, then the Notch1 proteins end
up in the daughter cell that begins migrating to its final
position, while the Numb proteins remain in the daughter
cell that stays in place and divides again. Notch1 therefore
seems to be the trigger for the genetic program that causes
cells to cease dividing and to migrate toward their final
locations. |
|
Most of the neuroblasts migrate over distances that are appreciable
when measured on the scale of the embryo. These distances range
from just a few millimetres (for cells migrating to the pia mater
in the primate cortex) to far greater distances (for cells migrating
from the neural
crest to the peripheral
nervous system).
Depending on their areas of origin and their destination, neuroblasts
use different methods to guide themselves during their migration.
The cells that come from the neural crests and migrate to the peripheral
nervous system, as well as those neurons that will form clusters
called nuclei in the brain, orient themselves chiefly by means
of cell
adhesion molecules. These molecules are located either in the
extracellular matrix or on the surface of other cells that these
migrating nerve cells encounter along their way. In addition, each
migration pathway thus determined provides opportunities for the
migrating neuroblasts to interact with various cell environments
that emit inductive signals which alter the neuroblasts and contribute
to their differentiation.
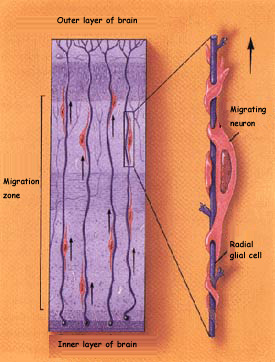 |
The other major method of migration
is observed in those brain structures where the cells stratify,
such as the cerebral
cortex, hippocampus,
and cerebellum.
In these structures, the neurons reach their final destination
by climbing along glial cells of a particular type, known
as radial glial cells. The migrating neurons use these glial
cells as highways and are pulled along them by the affinities
between the neurons’ own adhesion molecules and those
of the glial cells.
However, one-third of the neuroblasts
do not take this radial migration route, which can lead to
a certain horizontal dispersion of the cortical neurons derived
from the same precursor. |
The first neuroblasts that migrate from the venrtricular zone
are destined to form a layer called the cortical subplate,
which disappears in a later phase of development. The neuroblasts
that are destined to form the six
layers of the cerebral cortex then cross through this subplate
and form a new layer called the cortical plate.
The first cells to reach the cortical plate form layer VI in the
cortex; next come the cells that form layer V, then layer IV, and
so on, from the inside out.
As a result, the neurons that are born first are located in the
deepest layers of the cortex, whereas the younger ones are located
in the layers closer to the cortical surface. This migration guided
by the radial glia also provides an embryological explanation of
the columnar structure of the cortex. Each group of stem cells
in the ventricular zone naturally gives rise to a column of closely
interrelated neurons in the cortex.
Eventually, once the cortical neurons have reached their destinations,
the radial glial cells will retract their extensions. By the end
of the process of corticogenesis, the ventricular zone has become
nothing more than a single layer of ependymal cells that marks
the boundary of the cerebral ventricles.
Not all
neurons complete their migration successfully. In fact, the
experts believe that only one-third do so. The other neurons
either die and disappear during the two to three weeks that
the migration lasts, or they never differentiate,or they
survive and differentiate, but not in the right location.
This last group of cells may be the cause of various disorders,
ranging from learning disorders and dyslexia to epilepsy
and schizophrenia.
|
Each cell
individually is not required to undertake the entire journey
that leads from the activation of specific genes to the fulfilment
of this cell’s function in the organism. Many decisions
are made very early in development and throughout the differentiation
process. Because differentiation is a process that does not
reverse, the cells begin by activating genes responsible
for general functions of a given type of organ, but save
until the end the precise adjustments needed to place the
cell in its final position in the organ.
|
|
|